Abstract
Introduction
Materials & Methods
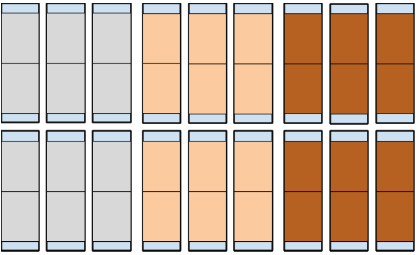
Figure 1. Experimental Design. Each large rectangle with thick outline represents a container. Within each container are two medium rectangles representing treated filter paper (light blue for distilled water, peach for unburned oil, brown for burned oil) that meet and slightly overlap in the middle of the container. At either end of each container are small rectangles representing the uncovered Vogel Agar that serves as the inoculation site. The top row of containers was inoculated with wild-type FGSC 2489 N. crassa and the bottom row was inoculated with mutant FGSC 13319 N. crassa.
Containers
Containers (large rectangles depicted in Fig. 1) were assigned their treatments. Half of the 18 containers were inoculated with wild type N. crassa FGSC 2489, and the other half were inoculated with FGSC 13319. Within each of these halves, 3 containers were assigned to a distilled water treatment, 3 were assigned to an unburned oil treatment, and 3 were assigned to a burned oil treatment, which was a mixture of unburned and burned oil in a 50:50 by volume ratio (the burned oil was diluted due to a lack of results in burned oil replicates in unreported pilot studies). There were two replicates within each individual container, for a total of 36 replicates, 6 within each treatment.
Filter Paper Treatment
For each container, two 7.62 cm x 12.65 cm filter papers (medium rectangles depicted in Fig. 1) were soaked with 1.5 mL of the assigned treatment. A micropipette was used to pipet 100 μL in a 5 x 3 grid pattern. Each 100 μL increment was separated by 2.54 cm (1.27 cm from edge of filter paper), with three increments across the width (7.62 cm) of the filter paper and five down its length (12.65 cm). Two filter papers were placed in each container so that the filter papers only slightly overlap in the middle. Each edge of the container is then left with 7.62 cm x 2.54 cm uncovered Vogel Agar (small rectangles depicted in Fig. 1), which served as the inoculation sites. This gridding pattern ensures that the filter papers are saturated and not oversaturated and that the amount of treatment is normalized. Unburned oil was Pennzoil 550035091 Motor Oil Lubricant. Burned oil was taken from a car after an oil change.
Inoculation
To inoculate, 100 μL of suspension (2.50 x 105conidia) was pipetted directly onto the middle of the edge where the 7.62 cm x 2.54 cm uncovered Vogel Agar and side of the container meet. Suspensions were vortexed between each inoculation.
Conditions and Measurements
The containers were stored in incubators set to 25 ºC, covered with parafilm. Parafilm was sterilized with 91% isopropyl alcohol. Growth was recorded and documented every 24 hours by measuring, with a ruler, the lateral appearance of macroscopic mycelial growth (in cm), relative to the edge of the filter paper nearest the inoculation site. Pictures were also taken of each individual replicate.
Results
The lateral growth was measured at 24 hour intervals, but only the data from the 72 hour interval was analyzed. Pictures were also taken every 24 hours. 72 hours after inoculation, there was no statistical difference between lateral growth across both strains of N. crassa (mutant (without hydrophobins) and wild type (with hydrophobins)) (two-way ANOVA, p > 0.05) (Fig. 2). Additionally, there is a difference between lateral appearance of mycelial growth across oil treatments (burned/unburned/control) (two-way ANOVA, p < 0.05) (Fig. 2). There was no interaction between oil treatment and fungal strain (two-way ANOVA, p > 0.05) (Fig. 2).
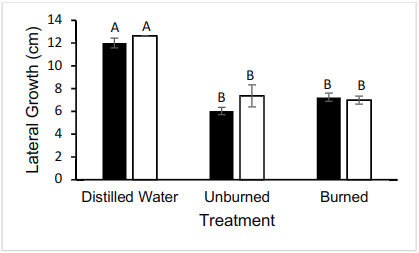
Figure 2. Lateral growth of N. crassa 72 hours after inoculation. Significant difference between distilled water (control) and either oil-based treatment. n = 6 for each treatment. Wild-type FGSC 2489 represented by black bars and mutant FGSC 13319 represented by white bars.
There is also no significant difference between Mutant Burned growth and Wild Type Burned growth (Tukey HSD p > 0.05), and Mutant Unburned growth was not significantly different from Wild Type Unburned growth (Tukey HSD p > 0.05) (Fig. 2).
Average Mutant Unburned was not significantly different from Mutant Burned growth (TukeyHSD p > 0.05) (Fig. 2). Average Wild Type Unburned growth was also not significantly different from Wild Type Burned growth (TukeyHSD p > 0.05) (Fig 2.).
Compared to the control replicates, which were grown in distilled water, both Mutant Burned and Mutant Unburned had significantly less growth (TukeyHSD p < 0.05) (Fig. 2). The same is true for Wild Type Burned and Wild Type Unburned compared to distilled water, each having significantly less growth (TukeyHSD p < 0.05) (Fig. 2). There was no significant difference between Mutant Control and Wild Type Control (p > 0.05) (Fig. 2).
In unburned treatments across both strains, the unburned oil spilled from the filter papers into the inoculation site. In burned oil treatments, there appeared to be localized concentrations of burned oil at the margin of hyphal growth (best seen in Figure 3, middle image). Burned oil treatments appeared to present with more fuzzy white macroscopic growth than the unburned oil treatments (Fig. 3).

Figure 3. Growth phenotypes. Images of wild-type FGSC 2489 in distilled water (A), burned oil (B), and unburned oil (C), as well as images of hydrophobin mutant FGSC 13319 in distilled water (D), burned oil (E), and unburned oil (F).
Discussion
Based on the results of this trial, burned and unburned oil significantly decreased the lateral growth of the mycelium and hydrophobin presence did not have an effect in any oil treatment. There was no significant difference in lateral growth between burned and unburned oil treatments.
Mutant growth was greater than wild type growth in unburned oil, but not significantly so. However, this is unlikely to be due to an actual effect of the lack of hydrophobins, as this difference mostly appears to be the result of a single high-growth outlier in the mutant unburned treatment. This outlier resulted in the relatively high error bars for the mutant unburned treatment. More replication is necessary to determine the validity of this outlier. Higher mutant growth in any oil treatment relative to wild type growth would also not be expected because the presence of hydrophobins serves to decrease surface tension at oil-water interfaces (Linder et al., 2005). So increased lateral growth in the absence of hydrophobins would contradict the current understanding of hydrophobins.
The interaction between the hydrophobic unburned oil and the water-based agar also led to a “flooding” effect that could have confounded the results in the unburned oil treatments. This could have affected the results as the inoculations would have immediately encountered the oil treatment, which has been shown in this study to impair growth. The burned oil treatments, which did not experience this “flooding,” did not encounter their treatment until they reached the filter paper.
The lack of a significant effect of hydrophobins on lateral growth was surprising, and so the strains were re-tested for “wettability” after the conclusion of the experiment to ensure that there had been no cross-contamination. There is no clear explanation for this lack of effect, and future studies should attempt to further characterize the role and impact of hydrophobins at hyphal tips exposed to oil.
Although there is no significant difference between unburned and burned oil, it is difficult to draw definitive conclusions about the effect of toxic compounds in the burned oil and their effect on the lateral growth. Firstly, the burned oil was diluted with unburned oil. The “flooding” effect in the unburned oil treatments must also be considered. Qualitative observations support this explanation, as the inoculation sites at 24 hours appeared to be covered with a film of unburned oil, but this “flooding” was not observed in the burned oil treatments. There also appeared to be a “pooling” of the dark pigment characteristic of the burned oil at the margin of hyphal growth in the burned oil replicates. It may be possible that the hyphae are somehow translocating the toxins concentrated in the burned oil that they are not capable of immediately degrading.
The significantly impaired lateral growth in the oil treatments, compared to the distilled water control treatments, could also be due to nutrient richness rather than only environmental impairment. The presence of massive hydrocarbons in these treatments could impede lateral growth as the fungi might prefer to grow a more heavily-branched mycelium when it encounters these large hydrocarbons in addition to the Vogel Agar. This could be a feasible explanation due to N. crassa having a relatively limited capacity for degrading crude oil (Ekundayo, F. O. et al., 2012). Although N. crassa can degrade crude oil, it may be that it cannot degrade crude oil to a degree that would be overall beneficial for its growth.
There is also an obvious effect on the composition of the mycelium due to burned oil exposure as observed by the dominance of the fuzzy white mycelial structures in these treatments. This change in appearance could possibly be related to the reproductive structures produced along the mycelium, and consequently this difference could have an effect on mycelial growth.
Future studies should attempt to observe and quantify the branching patterns when N. crassa is exposed to burned and unburned oil. Quantifying the relative amounts of the different reproductive structures of N. crassa when exposed to oil treatments could also be of interest. Higher replication and better control of the localization of the oil treatments in future trials would lead to more definitive results. Enzyme assays could be used to characterize the biochemical mechanism by which N. crassa breaks down the compounds in the oil treatments. A future study could then also attempt to increase the expression of relevant enzymes using genetic modification.
Any replicated trials of this experimental design should also seek to use a more concentrated burned oil solution. Here the burned oil was diluted to a 50:50 by volume ratio with unburned oil. This was done because pilot studies had found that the concentrated burned oil solution led to almost no growth of N. crassa. Future trials should attempt an 80:20 or 70:30 by volume ratio of burned oil to unburned oil.
Future research should also aim to further inform the role N. crassa could play in bioremediation. There must be a clear understanding of what byproducts are formed when introducing a bioremediative microorganism into a polluted environment, and so identifying the compounds produced as the result of N. crassa degradation of oil is important. Consideration must also be given to the other effects, independent of pollution, that a specific microorganism would have on the other populations and the abiotic environment composing the ecosystem into which it is introduced. Experiments growing N. crassa in an oil-contaminated environment that is also inhabited by other fungal species would be an example of a useful study for informing this concern.
Acknowledgements
We thank Dr. Kwangwon Lee for giving us the opportunity to carry out this project through his Principles and Practices of Biological Research (PPBR) course, as well as his generous gifts of Vogel Salts, N. crassa strains, culture tubes, and cell-counting cassettes.
We thank Dr. Nathan Fried for his role in teaching the PPBR course, as well as his assistance in generating ideas for our project, analyzing data, and providing feedback for our writing process.
We especially thank Ms. Sarah Johnson for answering all of our technical inquiries and for providing us with the materials necessary to make this project happen.
We thank Dr. Katie Malcolm and Dr. Jennifer Oberle for their helpful input based on their expertise with fungi.
References
Akintunde, W.O., Olugbenga, O.A., and Olufemi, O.O.(2015). Some Adverse Effects of Used Engine Oil (Common Waste Pollutant) On Reproduction of Male Sprague Dawley Rats. Open Access Maced JMed Sci 3, 46–51.
Bayry, J., Aimanianda, V., Guijarro, J.I., Sunde, M., and Latgé, J.-P. (2012). Hydrophobins—Unique Fungal Proteins. PLOS Pathogens 8, e1002700.
Ekundayo, F. O., Olukunle, O.F., and Ekundayo, E. A.(2012). Biodegradation of Bonnylight crude oil bylocally isolated fungi from oil contaminated soils in Akure, Ondo state. Malaysian Journal of Microbiology.
Linder, M.B., Szilvay, G.R., Nakari-Setälä, T., and Penttilä,M.E. (2005). Hydrophobins: the proteinamphiphiles of filamentous fungi. FEMS MicrobiolRev 29, 877–896.
Luke, A.K., and Burton, S.G. (2001). A novel application for Neurospora crassa: Progress from batch culture to a membrane bioreactor for the bioremediation of phenols. Enzyme and Microbial Technology 29,348–356.
Journal of Biological Sciences at Rutgers Camden (JBS) is licensed under a Creative Commons Attribution-NonCommercial-ShareAlike 4.0 International License