Sophie Cherfane, Nafisa Hasna, Waliya Rahman,
Alexis Ramirez Lopez, Syed Shamsi, and Christina Suoto
Department of Biology, Rutgers University, Camden NJ 08102
Peripheral nerve injuries are prevalent worldwide and can cause a range of impediments which may include, but are not limited to, loss of sensation and limited motor activity. Additionally, they can result in a slow and painful recovery process, calling for alternative treatment strategies. When a nerve injury occurs, the proximal and distal ends of the axon in the neuron take time to grow back. In recent years, different reagents such as magnesium have been explored to promote nerve regeneration and decrease recovery time. Magnesium is one of the most essential nutrients in metazoans. It plays a vital role in nerve regeneration and functional recovery by reducing inflammation and causing Schwann cell proliferation at the injury site, which increases axonal recovery. However, a lack of long-term studies persists regarding the effects of magnesium supplementation on motor recovery following a peripheral nerve injury. Here, we show that a magnesium supplemented diet implemented as either a prophylactic or therapeutic treatment does not play a significant role in motor function and nerve regeneration following a nerve crush injury in the fruit fly, Drosophila melanogaster. Our findings, however, indicate that the nerve crush injury paradigm is effective in successfully causing limited motor function due to dragging of the T3 pair of legs in flies. This shows that the nerve was injured successfully, demonstrating that the established method is a viable protocol for future groups to study functional recovery from nerve injuries in D. melanogaster. Our study demonstrates the necessity of further exploration of the mechanistic interactions between magnesium and its association with motor function and nerve regeneration. We anticipate that this research can act as a foundation to help test for alternatives in peripheral nerve injury treatments and in learning more about the role of magnesium following a nerve crush injury.
Abstract
Introduction
Peripheral nerve injuries are prominent, including more than 300,000 cases reported worldwide (Zhang et al., 2021). This makes it essential to understand the mechanism behind the neurological recovery process and potential therapeutic strategies, as such injuries can cause impaired motor activity, loss of sensation, and may result in lifelong disability (Zhang et al., 2021; Hussain et al., 2020). These injuries are generally classified into three categories: acute, subacute, and chronic, with subacute and chronic nerve injuries resulting from overuse of the injury (Radić et al., 2018). Overuse of an injury can result from repetitive movements and muscle strain, and is defined as conditions that cause discomfort or pain in areas such as muscles, joints, and nerves (Radić et al., 2018). Although the regeneration process is beneficial, it is very slow and can lead to complications such as limited recovery requiring patients to undergo other treatment options such as physical therapy and surgeries (Radić et al., 2018; Hussain et al., 2020).
Following a peripheral nerve injury, the injured neurons undergo a secondary oxidative stress response and degeneration, which leads to the production of several inflammatory cytokines, promoting the apoptosis of Schwann cells and Wallerian degeneration (Zhang et al., 2021). Wallerian degeneration is the process that takes place at the distal stump of an injured axon, where the myelin sheath breaks down into vesicles and collapses which causes secondary nerve damage (Wu and Murashov, 2013). The mechanism causes regeneration to be inhibited following a nerve injury and suppresses functional recovery. A long recovery time is often observed, as the proximal and distal ends of the axon take time to grow back due to the distance between the broken ends of the axon. However, the severed peripheral nerves do have the capability to regenerate and recover functional ability (Wu and Murashov, 2013). At present, nerve models made up of extracellular matrices have been used to provide mechanical guidance and a suitable microenvironment for nerve axon regeneration (Zhang et al., 2021). However, many of these reagents lack the ability to repair nerves that have a broken gap of more than 20 mm; hence, scientists have considered different minerals, such as magnesium, to use as a potential healing reagent. Due to magnesium’s protective effect on the nervous system, it has been used in recent years as a reagent to repair nerve damage (Zhang et al. 2021).
Magnesium plays a vital role in neurodevelopment. It is a foundation for signal transductions,
formation of myelin sheaths and synapses to proliferate signal transductions, and neurotransmitter communications. Recent studies have shown that magnesium can help aid neural axon growth (Zhang et al., 2021; Vennemeyer et al., 2014; Sun et al., 2012). Previous research has also been conducted to test the effects of magnesium as a treatment on both the central and peripheral nervous systems to treat damage done to the nerves (Kubiak et al., 2019; Hussain et al., 2020). However, the difference between how magnesium acts as a prophylactic, as opposed to a therapy for nerve regeneration and functional recovery following an injury, has yet to be analyzed.
We depict one hypothesized mechanism that factors into the function of magnesium in D. melanogaster as shown in Figure 1. Magnesium can bind to calcium channels, leading to the interactions with Bcl-2, a family of proteins that help inhibit apoptosis by preventing the release of cytochrome c, a mitochondrial protein used for cell death, and prevent a DCP-1 cascade that also leads to cell death (Dorstyn et al., 2002; Hwangbo et al., 2016). As a result, this leads to the production of NGF, or a nerve growth factor that regulates growth in neurons, thus further facilitating nerve regeneration and proliferation.
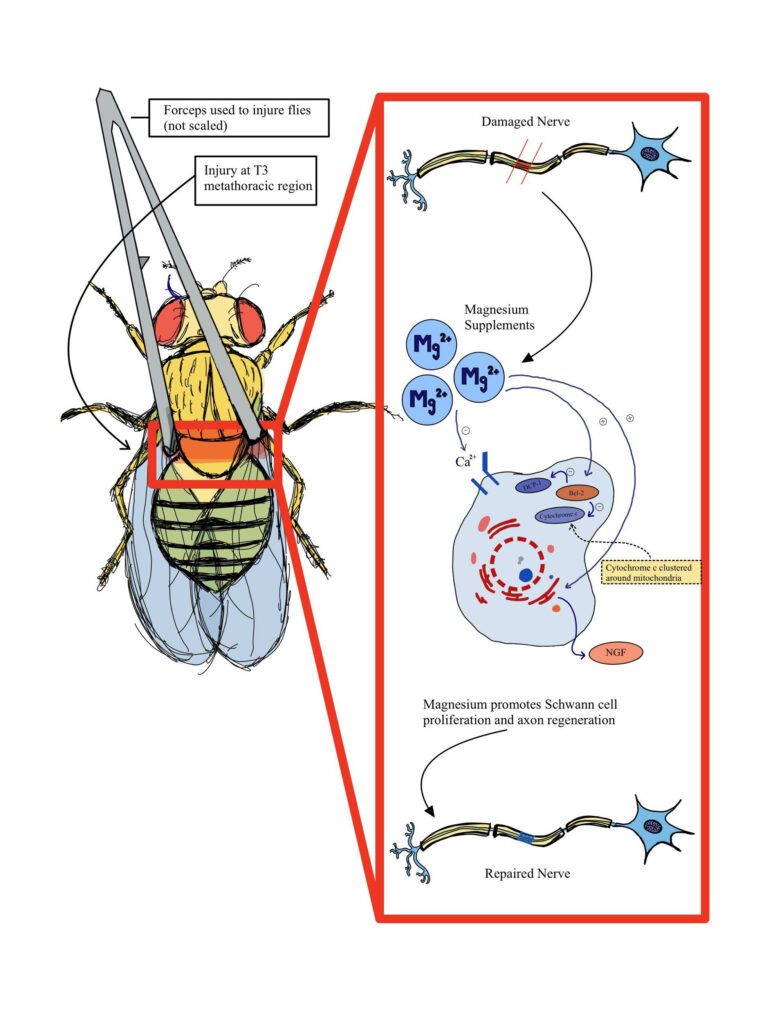
Figure 1. Diagram of hypothesized nerve recovery after injury assay performed on Drosophila melanogaster. Adapted from (Zhang et al., 2021).
Our study aimed to use Drosophila melanogaster to observe motor function as a proxy to analyze the extent of nerve regeneration of adult D. melanogaster following a nerve crush injury as a response to either magnesium administered as a prophylactic or a therapy. We hypothesized that a magnesium supplemented diet that is implemented as a prophylactic during the larval stage of the D. melanogaster would have greater peripheral nerve regeneration as opposed to the same diet as a therapy following a nerve crush injury. To test this hypothesis, the nerve crush injury paradigm was replicated by using forceps to apply pressure to the metathoracic segment of D. melanogaster adults one day after eclosion which led to the impaired movement of T3 legs (Losada-Pérez et al., 2021). This crush injury was performed on three groups of flies: the first served as a control and did not receive magnesium supplemented food, the second received a magnesium supplemented diet as a prophylactic from the larval stage, while the third group of flies received it as a therapy following the nerve crush injury. Since the injury was predicted to cause variations, the adult flies were observed for 15 minutes after the crush injury (ACI) to confirm dragging of T3 legs. These flies were then selected for the negative geotaxis assay, which was used to observe the motor movement of the flies days 1 and 3 after the crush injury since it takes the axons in the metathoracic segment of D. melanogaster about 8 hours to degenerate and in 24 hours axon regeneration begins with some axon debris found around the injury sites (Li et al.). The results showed that a magnesium supplemented diet implemented as a prophylactic and therapeutic treatment does not play a statistically significant role in motor function and nerve regeneration after an injury is inflicted. However, it was found that the injury paradigm was a viable way of causing limited motor function by successfully injuring the nerves connected to the T3 pair of legs in the D. melanogaster.
Materials & Methods
Fly Maintenance
Wild-type Oregon-R D. melanogaster flies were obtained from the Bloomington Drosophila Stock Center. The stock was cultured and maintained at room temperature (23ºC) and the flies were assigned to four experimental groups: a wing clipped control group, a wing clipped and injured control group, a therapy group, and a prophylactic group. Multiple trials were conducted for each experimental group, and different vials were used for every group, each containing 5-6 flies. Once the Oregon-R adult flies produced larvae, they were transferred to different vials to separate them from the other adults who did not emerge from feeding off of the experimental food. After eclosion, the period when the flies emerge from the pupal case, the new adults in each experimental group were collected and a nerve crush injury was performed on each other groups except for the control wing clipped only group. Following the injury, the flies were either transferred into a vial containing Ward’s Drosophila Medium White 4 L food or magnesium supplemented Ward’s Drosophila Medium White 4 L food based on the experimental group they belonged to
Experimental Groups
Four different groups of flies were observed in this experiment. The first group was designated as the wing-clipped control group, which consisted of flies that did not experience a nerve crush injury, and were fed Ward’s Drosophila Medium White 4 L throughout the experiment. These flies were used to get a baseline of how non-injured flies would perform on the negative geotaxis assay to compare with the injured flies and their performance in the assay. The second group was designated as the injured control group in which the flies were fed Ward’s Drosophila Medium White 4 L food during their larval stage. After eclosion, the flies were anesthetized, and a nerve crush injury was performed. The flies were then transferred into a vial with regular Ward’s Drosophila Medium White 4 L food. The same process was performed on the therapy group. However, this time, instead of transferring them into regular food following the nerve crush injury, they were placed into the magnesium supplemented food. The final experimental group consisted of flies in the prophylactic group, which were fed a magnesium supplemented diet from the beginning of their larval stage. Similar to the control group and the therapy group, after eclosion, these flies were anesthetized and a nerve crush injury was performed. The injured flies were then transferred to a new vial with a fresh supply of magnesium supplemented food.
Food Formulation
To make the 1 M MgCl solution, 4.7605 g of MgCl in powder form was massed and dissolved entirely in 50.0 mL of tap water. Once the solution was prepared, 8.0 mL of the solution was measured out as well as 42.0 mL of tap water to make a 160.0 mM stock solution of MgCl. Then A volume of 5.5 mL of 160 mM MgCl2 was added to 2.65 g of Ward’s Drosophila Medium White 4 L food, in addition to 2.0 mL of tap water to confirm sufficient consistency and ideal dosage of magnesium for the fly food.
Nerve Crush Injury
To perform the nerve crush injury a previously reported method of a nerve crush injury was adopted and modified for this specific experiment, as it enabled one to evaluate functional recovery since the cuticle and legs remain intact (Losada-Pérez et al., 2021). Flies were first anesthetized on ice packs and placed ventral side up, and immobilized using forceps. To minimize the metathoracic (T3) leg movement and locomotor dysfunction, forceps were used to exert pressure from the exterior to injure the metathoracic segment of adult flies by gently compressing the thorax. Since this injury was done by hand, there was a high possibility of variabilities to occur; hence, to limit these variations, only one person conducted the crush injury and identified injured flies 15 minutes after crush injury, selecting those who were dragging the third pair of legs. The wings of the flies were also clipped to prevent them from flying while performing the negative geotaxis assay to ensure that only their legs were prompting their motor function. The motor function and recovery of their legs were observed on day one and day three after the injury.
Negative Geotaxis Assay
During the negative geotaxis assay, an 8 cm benchmark was marked on the side of the vial to set the standard by which other groups within the experiment would be measured and compared. To perform the assay, ideally 10 qualified D. melanogaster ACI, based on the criteria set above, were placed in one enclosed vial and given 10 seconds to demonstrate their ability to cross that set benchmark. During this 10-second period, observations were made and averages were determined based on the number of flies that successfully crossed the benchmark. Between each trial, a 1-minute rest period was given to the flies for recovery before another trial could be performed; this was repeated a total of 10 times (Ali et al., 2011). In order to reduce variability and ensure that the flies did not use their wings to fly across the benchmark, their wings were clipped prior to performing the assay. The clipping of the wings would allow for any observations of functional recovery to be made solely on how well their legs recovered functional mobility. This assay was performed on day 0 (the day of injury), day 1, and day 3 after the injury to analyze the change in motor function of the flies in each of the experimental groups.
Pilot Study
A pilot study was conducted to observe the effectiveness of the nerve crush injury on D. melanogaster as they performed the negative geotaxis assay. Four wild-type D. melanogaster were placed into two separate vials and fed Ward’s Drosophila Medium White 4 L food. They were designated as two groups: wings clipped without an injury, and wings clipped with a nerve crush injury. After a period of 24 hours, the assay was performed and the two groups were compared; these groups of flies were timed for 10 seconds as they climbed up the vials crossing the 8 cm benchmark, and the average number of flies that crossed the line in 10 trials was recorded. Data from this particular study allowed for the examination of statistically different results for the non-injured and injured flies following the negative geotaxis assay and confirmed the efficacy of the nerve crush injury.
Results
A pilot study was conducted to test the viability of the nerve crush injury to determine if it had an impact on the motor behavior between the injured and non-injured flies during the negative geotaxis assay. To accomplish this, the metathoracic segments of the D. melanogaster flies were crushed and flies with noticeable leg drag were selected (Ali et al., 2011). The negative geotaxis assay was performed on the allocated groups 24 hours ACI to measure their ability to climb. The assay was replicated 10 times to calculate an average value for the flies that were able to cross the 8 cm mark. As anticipated, flies with the nerve crush injury had a lower success rate of climbing past the benchmark within 10 seconds compared to the flies without the nerve crush injury. It was observed that an average of 80% of flies that were wing clipped were able to climb the vials successfully, while the flies that were wing clipped and injured, only an average of 15% were able to do so (Fig. 2). The findings of this pilot study revealed a statistically significant difference between the Wings Clipped only and Wings Clipped with a nerve crush injury group indicating that the procedural nerve crush injury performed was effective in injuring the peripheral nerve of the flies (t-test; p* < 0.05).
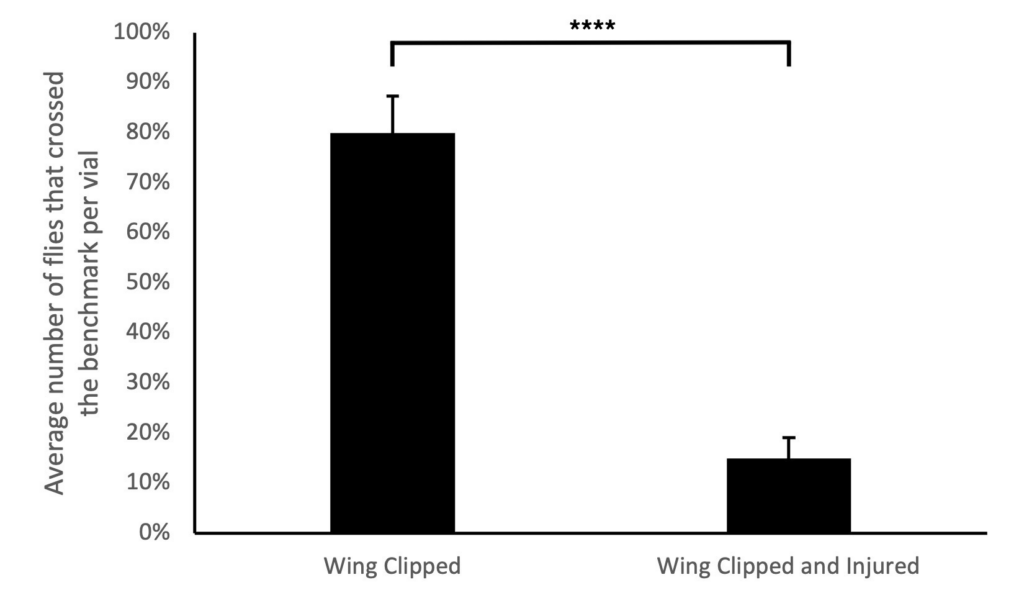
Figure 2. Pilot study reveals that the nerve crush injury was an effective method of injuring the D. melanogaster flies to observe functional recovery since it reduced the negative geotaxis assay success when performed 24 hours after the injury. The average percentage of the two experimental replicates with a total of 8 flies in each replicate that crossed the 8 cm benchmark in 10 seconds was demonstrated. The assigned replicates were: flies with wings clipped only (n=4) and flies with wings clipped with a nerve crush injury inflicted (n=4). The error bar represents one standard error. The average of flies with injury and their wings clipped crossed the benchmark significantly less than flies with their wings clipped only (t-test; p* < 0.05).
To determine if magnesium supplementation increases nerve regeneration following a nerve crush injury, the motor behavior of the four experimental groups: Control Wing Clipped, Control Wing Clipped & Injured, Therapeutic Wing Clipped & Injured, and Prophylactic Wing Clipped & Injured, were compared to each other via the negative geotaxis assay over three days (Fig. 3). No significant differences were observed between the four different experimental groups (repeated ANOVA; p > 0.05, bonferroni; p > 0.05). A comparison of Day 1 and Day 3 resulted in a statistically significant difference between the Prophylactic Wing Clipped and Injured group and Therapeutic Wing Clipped and Injured group (repeated measures ANOVA; p* < 0.05, bonferroni; p < 0.05). However, the Control wing clipped, Prophylactic, and Therapeutic wing clipped and injured groups had the same trends, and only the Control wing clipped and injured group had a different trend than the other three groups (Fig. 3). Overall, it was determined that there was no significance between the four groups (two-way ANOVA; p > 0.05, with post hoc t tests, p > 0.05).
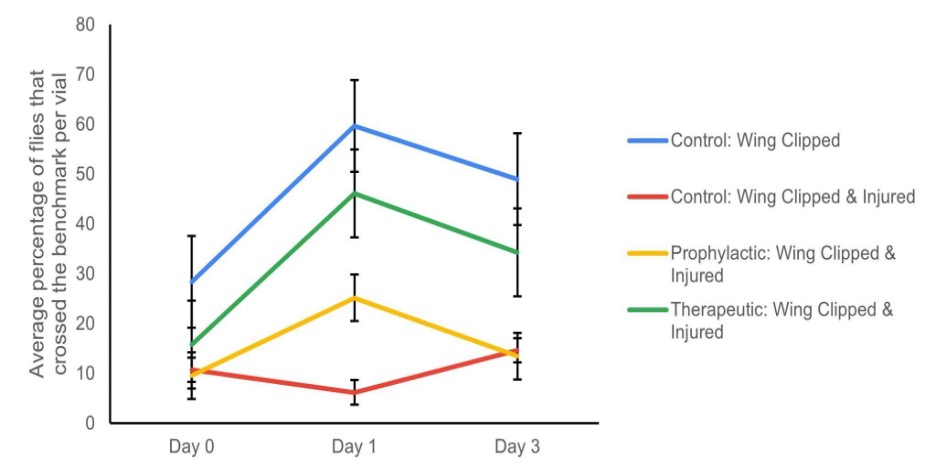
Figure 3. Averages of the success rate of the wild-type Oregon-R D. melanogaster flies in the negative geotaxis assay. The experimental data consisted of three different sets of trials. The percentage of the experimental groups (Control: Wing clipped, Control: Wing clipped & Injured, Therapeutic: Wing clipped & Injured, and Prophylactic: Wing clipped & Injured) that crossed the 8 cm benchmark in 10 seconds ACI on Days 0 (the day of injury), 1 and 3 in these three trials is illustrated (n=3). The error bar represents one standard error. There was no significance between the four groups over the 3 days (two-way ANOVA; p > 0.05, with post hoc t-tests, p > 0.05).
Discussion
This study aimed to determine whether a magnesium supplemented diet that is implemented as a prophylactic during the larval stage of the D. melanogaster or administered as a therapeutic following a nerve crush injury will result in improved peripheral nerve regeneration. This was indirectly measured by observing the functional recovery of the flies using the negative geotaxis assay success rate ACI, to understand the role of magnesium on nerve regeneration. We hypothesized that the prophylactic group would have improved functional recovery over a three-day span after a nerve crush injury is performed compared to the therapeutic group because magnesium plays an important role in the enhancement of the cellular mechanisms that are responsible for regeneration (Zhang et al., 2021; Vennemeyer et al. 2014; Sun et al., 2012). Therefore, it was predicted that the prophylactic group of flies will have a better response to an injury due to the excess magnesium within their cellular systems that can be utilized in the nerve regeneration process. Also, the therapeutic group was predicted to perform slightly less than the prophylactic group because they will receive the magnesium supplemented diet only directly following an injury.
To reduce variation and keep the level of injury consistent for each trial, one person was put in charge of performing the injury for one trial at a time. Another area of variation was addressed by placing the flies in an empty vial in a horizontal position as they awoke from anesthesia. This strategy helped us ensure that they were not getting stuck in the food and were able to move properly, yielding a more standardized total number of flies to run the experiments. In future studies, such variations could be limited by conducting the experiment on a higher number of flies for a prolonged period to collect more data and analyze results.
Given limited resources, we were not able to examine the nerve recovery of the flies in detail using microscopy and could not fully explore the hypothesized mechanism in Figure 1. Therefore, fly behavior after the injury was used to determine if the nerve injury was performed successfully and whether behavior changed after supplementing magnesium to the flies diet the days after the injury. However, despite careful selection and utilizing steps to decrease fly mortality rates, many would either succumb to their injuries or could not correct themselves once they landed on the fly food media and would then suffocate. Due to this, after analyzing the data, we found our data to be statistically non-significant. Thus, we failed to reject our null hypothesis because according to the statistical results, a magnesium supplemented diet did not demonstrate improved motor function and nerve regeneration following a nerve crush injury in D. melanogaster.
Nevertheless, this study was valuable for further delving into the impact of mineral supplementation on nerve regeneration following a peripheral nerve injury. Specifically, it focused on magnesium and its mechanistic approach to heightening nerve regeneration. The outcome of this current study revealed that magnesium supplementation being administered as a prophylactic or therapeutic does not show increased functional recovery, which was used as an indicator for nerve regeneration. Although the null hypothesis was not successfully refuted, future long-term research could focus on nerve regeneration at the microscopic level to try and develop an understanding of the supplement of interest as preventative medicine for peripheral nerve injuries. Given the adapted method of observing functional recovery after a nerve crush injury, exploring the effects of other supplements with the necessary tools to visualize their effects, would be considered a logical next step. Based on literature however, some gaps have yet to be filled in terms of identifying a thorough and complete understanding of a mechanism responsible for nerve regeneration and its potential ties to magnesium. Taking this into consideration it can be determined that our research would benefit best if followed by the implementation of microscopy. Microscopy could be integrated with the intent of allowing the researchers to analyze components that were beyond what could be observed from the exterior of the Drosophila. Through this tool, neural regrowth or regeneration, if any, can be identified and recorded through the observation of target nerves in flies that can express the green fluorescent protein (GFP) (Soares et al., 2014). This would serve as a strategic way to spot axonal growth at the injury site which coincides with existing neural regrowth.
Overall, peripheral nerve injuries affect many individuals worldwide, with devastating consequences that include debilitation and long recovery periods. The prominence of such injuries within our society reiterates the importance of looking into components of this research and its potential influence on patients in the future.
Acknowledgments
We would like to express our gratitude to Dr. Kwangwon Lee and Dr. Nathan Fried for the support, comments, and suggestions they provided throughout the duration of the project. Additionally, we appreciate Mr. Adam Poff and Ms. Sarah Johnson for their tremendous contributions to arranging the supplies and guidance we required during the process. Finally, we would like to thank Rutgers University – Biology Department for providing us with lab space and funding.
References:
Ali, Y.O., Escala, W., Ruan, K., and Zhai, R.G. (2011). Assaying Locomotor, Learning, and Memory Deficits in Drosophila Models of Neurodegeneration. Journal of Visualized Experiments : JoVE https://doi.org/10.3791/2504.
Dorstyn, L., Read, S., Cakouros, D., Huh, J. R., Hay, B. A., & Kumar, S. (2002). The role of cytochrome c in caspase activation in Drosophila melanogaster cells. The Journal of Cell Biology, 156(6), 1089–1098. https://doi.org/10.1083/jcb.200111107
Farsi, L., Afshari, K., Keshavarz, M., NaghibZadeh, M., Memari, F., and Norouzi Javidan, A. (2014). Postinjury treatment with magnesium sulfate attenuates neuropathic pains following spinal cord injury in male rats. Behavioural Pharmacology 26. https://doi.org/10.1097/FBP.0000000000000103.
Hussain, G., Wang, J., Rasul, A., Anwar, H., Qasim, M., Zafar, S., Aziz, N., Razzaq, A., Hussain, R., de Aguilar, J.-L.G., et al. (2020). Current Status of Therapeutic Approaches against Peripheral Nerve Injuries: A Detailed Story from Injury to Recovery. Int J Biol Sci 16, 116–134. https://doi.org/10.7150/ijbs.35653.
Hwangbo, D.-S., Biteau, B., Rath, S., Kim, J., & Jasper, H. (2016). Control of apoptosis by Drosophila DCAF12. Developmental Biology, 413(1), 50–59. https://doi.org/10.1016/j.ydbio.2016.03.003
Kubiak, C.A., Kemp, S.W.P., Cederna, P.S., and Kung, T.A. (2019). Prophylactic Regenerative Peripheral Nerve Interfaces to Prevent Postamputation Pain. Plastic and Reconstructive Surgery 144, 421e. https://doi.org/10.1097/PRS.0000000000005922.
Li, Dan, et al. “A Drosophila In Vivo Injury Model for Studying Neuroregeneration in the Peripheral and Central Nervous System.” JoVE (Journal of Visualized Experiments), no. 135, May 2018, p. e57557. www.jove.com, https://doi.org/10.3791/57557.
Losada-Pérez, M., García-Guillén, N., and Casas-Tintó, S. (2021). A novel injury paradigm in the central nervous system of adult Drosophila: molecular, cellular and functional aspects. Dis Model Mech 14, dmm044669. https://doi.org/10.1242/dmm.044669.
Radić, B., Radić, P., and Duraković, D. (2018). PERIPHERAL NERVE INJURY IN SPORTS. Acta Clin Croat 57, 561–569. https://doi.org/10.20471/acc.2018.57.03.20.
Soares, L., Parisi, M., & Bonini, N. M. (2014). Axon Injury and Regeneration in the Adult Drosophila. Scientific Reports, 4, 6199. https://doi.org/10.1038/srep06199
Sun, Y., Zhang, B., Wang, Y., Geng, L., and Jiao, X. (2012). Preparation and characterization of a new biomedical Mg–Zn–Ca alloy. Materials & Design 34, 58–64. https://doi.org/10.1016/j.matdes.2011.07.058.
Vennemeyer, J.J., Hopkins, T., Kuhlmann, J., Heineman, W.R., and Pixley, S.K. (2014). Effects of elevated magnesium and substrate on neuronal numbers and neurite outgrowth of neural stem/progenitor cells in vitro. Neurosci Res 84, 72–78. https://doi.org/10.1016/j.neures.2014.05.001.
Wu, D., and Murashov, A.K. (2013). Molecular mechanisms of peripheral nerve regeneration: emerging roles of microRNAs. Front Physiol 4, 55. https://doi.org/10.3389/fphys.2013.00055.
Zhang, J., Zhang, B., Zhang, J., Lin, W., and Zhang, S. (2021). Magnesium Promotes the Regeneration of the Peripheral Nerve. Frontiers in Cell and Developmental Biology 9.
Journal of Biological Sciences at Rutgers Camden (JBS) is licensed under a Creative Commons Attribution-NonCommercial-ShareAlike 4.0 International License