Sarah A. (Mullins) D’Angelo, Kiyoshi Woods, and John Crespo
Department of Biology, Rutgers University, Camden, N.J. 08102
Abstract
Acute pain is widely accepted as having evolved to be an adaptive mechanism intended to immediately protect an organism from further harm following tissue damage or injury. Chronic pain, on the other hand, has historically been categorized as a solely maladaptive mechanism with no beneficial purpose. However, as science advances, the previous validity of chronic pain’s role within the pain field needs to be reevaluated. Recent studies have suggested that chronic pain-induced hypervigilance evolved to protect an organism when the initial acute response failed to protect it from initial injury by increasing awareness of potential predators within the environment. This study seeks to explore if chronic pain-induced hypervigilance could be a protective adaptation to increase survival rates in Drosophila melanogaster in response to a novel environmental danger paradigm. Painless D. melanogaster are genetically engineered to not feel pain and are used in this study to compare the survival rates of a group that experiences chronic pain (WT+A) and a group that will not be able to experience this mechanism (PL+A). Here, we show that wild-type Drosophila experiencing chronic pain from a leg amputation have significantly higher survival rates than the PL+A drosophila.
Introduction
The perception of acute pain exists as a sensory modality that directs attention to an injury to prevent further harm. Unlike acute pain, which is widely accepted to be a protective mechanism, chronic pain has long been described as a solely maladaptive process (Walters and Williams, 2019). Only recently has it been proposed that chronic pain may have evolved as an adaptive mechanism (Neely et al., 2019; Mogil et al., 2020), which challenges the fundamental understanding of chronic pain.
According to the smoke detector principle (SDP), physical responses such as inflammation, anxiety, and pain evolved as biological mechanisms to prevent further or potential harm that would be catastrophic to the organism if not avoided (Nesse, 2019). The SDP posits that so-called “false alarms” or excessive reactions are a necessary component for survival because the costs of the absence of such reactions far outweigh the consequences brought on by the over-active reactions themselves.
Nociception or the detection of harmful (i.e., painful) stimuli is a sensory process key to survival for any living being. The term nociception is often used in discussing pain in animals due to pain’s complex, and oftentimes subjective nature. The importance of nociception has been demonstrated in humans born with congenital insensitivity to pain (CIP) who cannot feel the sensation of pain. Morbidity and mortality rates are significantly higher in documented cases of people with the permanent inability to feel pain, and most do not survive past their twenties (Costigan et al., 2009; Hwang et al., 2007).
Recent studies have proposed that hypervigilance caused by nociceptive sensitization might have acted as a natural selective pressure for evolution to increase Darwinian fitness (Kavaliers, 1988; Lister et al., 2020). However, the neuroethological mechanisms behind this response are still largely unknown and have yet to be explored. The studies that have investigated nociceptive responses in adult D. melanogaster, mostly report the use of thermal stimuli (i.e. UV irradiation and hot plates) (Hwang et al., 2007; Xu et al., 2006) to induce tissue damage leading to sensitization but do not directly measure chronic pain’s impact on survivability. It is well established that D. melanogaster larvae sensitize in response to thermal, mechanical, and chemical tissue damage suggesting polymodal mechanisms utilized for intervertebral nociception, comparable to more neurologically complex organisms (Im and Galko, 2012). Still, it has not yet been understood if and how adult D. melanogaster produces similar sensitization to mechanical sensory modality. Recently, a study has shown that nerve damage increases sensitization and hypervigilance in adult D. melanogaster. (Khuong et al., 2019).
In this study, we seek to investigate chronic pain’s possible adaptive significance, inspired by (Lister et al., 2020) and (Crook et al., 2014) publications, which explored chronic pain’s evolutionary mechanisms as they relate to hyperexcitability and behavior in rodents and squids, respectively. We are branching off from the current literature by using invertebrates that are considered to be of lower order biologically (adult D. melanogaster) to investigate how hypervigilance, induced by chronic pain influences survival rates when exposed to the threat of external harmful stimuli in Ore-R Wild-type (WT) and painless gene (PL) D. melanogaster (experimental groups shown in Fig. 1A). Here, we produce a novel paradigm to assess the influence chronic pain has on survivability when faced with an external, harmful environmental pressure. We suspect that biologically induced hypervigilance initially arose as a mechanism to avoid predation but likely expanded to a more generalized state of heightened awareness of the surrounding environment.
Current literature has examined the role of chronic pain in a species’ survival when encountering a predator (Crook et al., 2014) and how chronic pain makes an animal more hypervigilant to a predator-prey paradigm (Lister et al., 2020). Therefore, we are branching off literature by using an environmental paradigm in which chronic pain will be studied in a form outside of a predator encounter.
We hypothesized that injured Ore-R WT D. melanogaster (WT+A) with chronic pain-induced hypervigilance caused by nerve damage from a limb amputation (see Fig. 1B) will display higher survival rates than injured painless gene D. melanogaster (PL+A). This is because the injuries that were sustained from the WT+A group will cause the organism to feel constant pain, causing the D. melanogaster to be hyper-aware of their surroundings, thus giving them a greater chance of survival when in the presence of immediate danger. Here, we show that the WT+A group likely had higher survival due to chronic pain-induced hypervigilance that alerted them of the danger of the hot temperature present in a water bath, allowing them to act quick enough to escape (climb to the top of the Petri dish where the temperature was less harmful) before mortality occurred. There should be little to no variation between the control (non-amputated WT) and experimental control (non-amputated painless) groups since neither will be injured by amputation. To test this hypothesis, our research project explored chronic pain’s neuroethological adaptive significance in the context of Darwinian fitness by using a thermal assay with Ore-R WT and painless gene D. melanogaster being put in a hot water bath.
Materials and Methods
D. melanogaster Lines and Maintenance
The painless gene D. melanogaster (fruit flies) were ordered from BDSC (Bloomington Drosophila Stock Center; stock number 27895). The Oregon-R (Ore-R) wildtype were generously provided by Dr. Lee’s lab.
Ward’s Ⓡ Instant Drosophila medium was used to feed all the D. melanogaster throughout the study. Five vials were pre-prepared utilizing a one-to-one ratio of distilled water and dry instant Drosophila mix. Each food vial was labeled with the creation date, name of the individual who made the mix, and “PPBR”, then refrigerated to ensure freshness. D. melanogaster of both male and female sexes were kept out of direct sunlight at 25°C under a 12-hour/12-hour light/dark cycle. All trials were performed at dusk.
Data Analysis
Guided by the findings of previous literature demonstrating injury-induced behavioral sensitization (Crook et al., 2014; Hwang et al., 2007; Khuong et al., 2019; Nesse, 2019), the injured amputated limb WT group would display higher survival rates than the injured painless gene amputated limb group. There should be little to no variation between the control (WT) and experimental control (PL) groups since neither will be injured by ligation.
Survival rate percentages were calculated by counting the D. melanogaster using point observations of the number of D. melanogaster remaining alive following the thermal assay divided by the number of initial D. melanogaster (n=10) and multiplied by 100 to gain the percent:
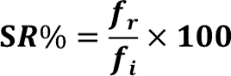
where SR%= Percent survival rate of D. melanogaster, fi= initial number of D. melanogaster, and fr= number of remaining D. melanogaster.
To test for a significant difference among our samples, T-tests, a full ANOVA, followed with Tukey’s post hoc test was utilized. For the ANOVA test, the groups column was the independent variable and the survival rate column was the dependent variable. This was done with the use of RStudio.
Pilot study
Before we could compare the effects of chronic pain among the 4 groups, we needed to validate the thermal assay itself. We wanted to find a standardized time that can repeatedly deliver the same mortality rate among our control to be able to highlight the effect that chronic pain has in this environmentally dangerous paradigm. We were able to determine this time point and validate this novel paradigm using a control group of WT D. melanogaster (n = 10) and finding the time point that returned a consistent 50% mortality rate. The pilot thermal assay had its first mortality at the 250-second mark, totaling 2 D. melanogaster mortalities. The experiment was then run at a longer duration to reach the 50% mark. This was then recorded to be at 400 seconds of the D. melanogaster being exposed to the 46° C heat chamber. The process was repeated starting at this new point of 400 seconds to ensure that it was a valid baseline time point. The final time point was found to be 6 minutes and 45 seconds. This was repeated twice with a new batch of D. melanogaster each time with a 50% kill rate for each test.
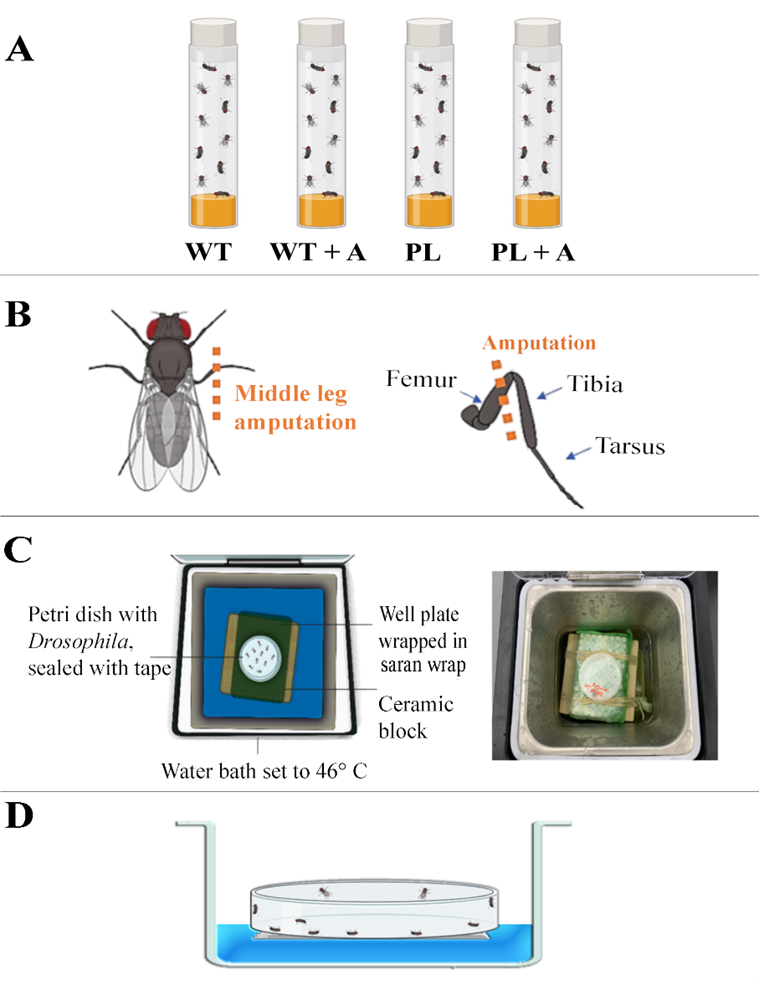
Fig. 1: Experimental Groups, Amputation Injury Model, Design of the top view of Thermal Assay, Side View of Thermal Assay. (A) Experimental Groups: Uninjured Positive Control (WT): Ore-R WT D. melanogaster with no amputated limbs, Injured Ore-R WT D. melanogaster with one amputated (middle) limb (WT+A), Uninjured Negative Experimental Control: painless gene D. melanogaster with no amputated limbs (PL), Injured painless gene D. melanogaster with one amputated (middle) limb (PL+A). (B) Amputation Injury Model. Each experimental trial utilized new stocks of D. melanogaster. Seven days before the thermal assay was conducted, two sets of 10 D. melanogaster (P+A group and WT+A group) were lightly anesthetized on ice before using micro-vannas scissors to perform the amputation of the right middle leg at the femur segment with the D. melanogaster laying prone. (C) Design (left) and Photograph (right) of top view of Thermal Assay. Each group of D. melanogaster were exposed to a water bath thermal assay with a constant temperature of 46° C. This thermal assay consisted of a water bath at a constant temperature of 46° C containing the petri dish holding the D. melanogaster on top of a well plate wrapped in saran wrap on top of a ceramic block acting as a platform. The D. melanogaster were placed in a petri dish with Scotch tape covering the exterior to prevent humidity from forming within the enclosure as well as to avoid the possibility of D. melanogaster from escaping the Petri dish. (D) Design of side view of Thermal Assay Showing 50% D. melanogaster Death. After given 30 seconds to rest, the petri dish was placed back into the water for an increase of 5 seconds and repeated until the sample size reached a mortality rate of 50% (n = 5) of the original sample (n = 10).
Results
To follow up with the pilot study that was conducted, a similar procedure was utilized to conduct our experiment. Alternatively, the thermal assay was used to look for the survival rate of each individual group after the 400-second mark rather than testing mortality at 50%. Testing for the survival rate allows us to compare the dependence of survival of each group.
As shown in Fig. 2, the WT had an average survival rate of 73.58 % with a standard deviation of ± 17.39, WT+A with 42.55% with a standard deviation of ± 5.40, PL with 57.74% with a standard deviation of ± 6.84, and the PL+A group with a survival rate of 15.87% and a standard deviation of ± 5.40.
In analyzing the difference in survival between all groups using the ANOVA test in addition to Tukey’s HSD, the comparison between WT+A – PL had the highest p value (p>0.05) which showed that there was no significant difference between the comparison of these two groups. In addition, the comparison between WT-PL had a p value that was above the 0.05 mark, which also indicates that there is no significant difference. However, the other groups did have a difference amongst each other. Where WT+A – PL+A had the highest p value (p<0.05). With the p value decreasing with the comparison of PL+A – PL (p < 0.005), WT+A – WT (p <0.0005), and then WT – PL +A (p < 0.00005) having the most significant difference. Which this is also supported by the bar graph illustrating the comparison between the survival rates of all groups (Fig.2).
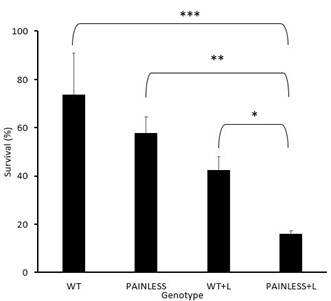
Fig. 2: Heat assay experimental data among 4 groups of D. melanogaster. The data represents the results from each group of D. melanogaster used for the heat assay behavioral experiment. The water bath was kept at a constant temperature 46°C. The time to achieve a 50% death rate was found to be 6 minutes and 45 seconds, confirmed with three pilot trials. Figure 2 illustrates the comparison between the four groups, indicating survivability depending on genotype (Tukey’s HSD, * p<0.05, ** p<0.01, *** p< 0.001)
Discussion
In this study, we were mainly interested in comparing differences in survivability among the injured groups. We predicted that all uninjured groups would have significantly higher percentages of survival, so it came as no surprise that both the WT and the PL uninjured groups had fewer mortalities compared to the injured WT+A and PL+A groups. In the comparison of the painless with amputation and the WT+A, it became clear that the WT+A group had significantly higher survival rates than the PL+A groups.
These results suggest that increased awareness brought on by the nerve damage from the removal of a limb allowed the WT+A group to have an increased capacity to react to the harmful stimuli of dangerously hot temperatures significantly faster than the PL+A group, ultimately enabling the organism to avoid death. The PL+A group of D. melanogaster were likely not able to detect the harmful stimuli fast enough to make the life-saving decision of escape due to their genetic inhibition of pain sensation (Xu et al., 2006). The findings ultimately strengthen the concept that chronic pain serves as an adaptive mechanism in the survival of D. melanogaster in a potentially dangerous environment
Thus, chronic pain may have been a natural selective pressure for the evolution of an extra line of protection for organisms through increased awareness of external stimuli and the environment. It is plausible that after tissue damage has occurred, the memory of the painful experience is stored within the organism’s neural processing system and is retrieved when the organism encounters similar dangerous environments. The lasting injury also likely causes an organism to be hyper-aware of its surroundings as to not cause further harm.
It may also be assumed that in the case of increased survivability due to chronic pain that in the comparison of the WT and WT+A groups, the WT+A group should have higher survivability. However, this is not the case. Previous literature describes a phenomenon of “cost of injury” in that an injury is costly to an animal and can cause a higher chance of predatory encounters, even if no noticeable changes in the animal’s behavior can be noted (Crook et al., 2014). Interestingly, by looking at groups affected by anesthetic treatment at the time of injury, groups that were anesthetized have the highest rate of mortality compared to those that experienced pain. This revealed that the full cost of injury is partially compensated by sensitized behavior(Crook et al., 2014). This can be compared to an animal running from a predator. An animal that has no broken limbs will have the highest chance of survival (WT), while an animal that has a broken limb (WT+A) will have a lower chance of survival due to the cost of injury associated with the broken limb. Results from our experiment are supported by previous literature that having a group with an injury will still have a lower survival rate than an unharmed group.
Historically, the hypervigilance associated with chronic pain has been thought of as only occurring in organisms classified as being of utmost neurological complexity (Crook et al., 2014; Lister et al., 2020). Our study, however, provides evidence that this aspect of chronic pain is also seen in a so-called “lower-order” organism, suggesting a survival mechanism associated with chronic pain in a wider variety of organisms. Furthermore, our study indicates that chronic pain serves an adaptive purpose to increase Darwinian fitness in lower ordered species, in this case, D. melanogaster, a concept previously only explored in higher ordered animals such as mammals (rodents) (Lister et al., 2020) and neurologically complex invertebrates (squid) (Crook et al., 2014). In addition to chronic pain having a vital role in a broad spectrum of organisms, including those of lower order, our study provides a novel paradigm for studying how chronic pain influences an organism’s decision-making processes of evaluating risk versus reward. This study elucidates the idea of chronic pain-induced hypervigilance succeeding to enhance awareness for any harmful stimuli, not just in a presence of a predator (Crook et al., 2014; Lister et al., 2020). Using an environmental stimulus that presents the threat of imminent danger leading to potential death paves the way for hypervigilance-induced chronic pain to be explored in ways that go beyond the traditional predator-prey paradigm.
Results from this experiment yield further evidence supporting the notion that our fundamental conceptualizations and understandings of the inner workings of pain desperately need to be reexamined. Unambiguously, acute pain serves an invaluable role in protecting an organism by triggering an immediate escape response when confronted with tissue damage (Walters and Williams, 2019). But studies such as this point to the possibility that chronic pain played just as, if not more of, an important role in survival through the induction of hypervigilance when the initial escape responses failed to protect the organism from harm. When an injury has already occurred, sensitization throughout the organism’s nervous system is triggered through neuroplastic mechanisms that remain to be fully understood. Further studies are needed to fully appreciate the bidirectional modality of chronic pain and hypervigilance.
As previously mentioned, humans with CIP are born with a significant disadvantage in fitness compared to those with the ability to recognize painful stimuli (Costigan et al., 2009). Their inability to both experience and perceive pain impedes their capacity to take protective measures that would allow them to avoid further harm once initial tissue damage has occurred. Similar to humans with CIP, the injured painless gene D. melanogaster utilized in our study had a significant disadvantage in survivability when faced with the external threat of imminently lethal tissue damage brought on by the thermal assay. Perhaps, this novel environmentally hazardous paradigm could be utilized with D. melanogaster as models for CIP in humans. Understanding the complex mechanisms responsible for the inability to feel pain along with how chronic pain may have evolved as a biological pressure to increase survival through enhanced hypervigilance could be promising for future CIP therapeutics. Given the extremely high mortality and morbidity rate seen in humans with CIP, it would be worth exploring this often-over-looked subset of the population.
There is still much to be learned about the many complexities of chronic pain, but studies such as this will hopefully help pave the way towards a greater understanding of not just chronic pain in D. melanogaster but also begin to unravel the many mysteries of the intricate human experience of chronic pain. More empirical evidence supporting the existence of a multitude of complex pain-associated behaviors linked to sensory experiences is deeply warranted. However, the more we understand how chronic pain evolved, and how many times it diverged to be the way it is in the present day, the closer we can get to finding novel approaches in pain management and treatment. Ultimately, knowledge gained from studies such as this will contribute to research that eventually provides much-needed relief to the plethora of humans across the world suffering from debilitating chronic pain conditions.
Studying chronic pain in an evolutionary context may not have immediately obvious implications for human health, especially when using models of lower ordered organisms such as D. melanogaster. Pain is an innately negative experience, with relief from said pain being intrinsically rewarding. Most preclinical models of chronic pain continue to focus solely on discovering mechanisms to alleviate the burden of or extinguish the perception of pain entirely. It has been clearly established that nociception is indeed a key player in the survivability of an organism, regardless of its neurobiological complexity. Perhaps, shifting the focus from solely eliminating chronic pain to trying to understand why chronic pain evolved to be the way it is today could be a key to bridging gaps in both translation and knowledge that is so desperately needed in the field of pain research.
References
Costigan, M., Scholz, J., and Woolf, C.J. (2009). Neuropathic Pain: A Maladaptive Response of the Nervous System to Damage. Annu. Rev. Neurosci. 32, 1–32.
Crook, R.J., Dickson, K., Hanlon, R.T., and Walters, E.T. (2014). Nociceptive Sensitization Reduces Predation Risk. Curr. Biol. 24, 1121–1125.
Hwang, R.Y., Zhong, L., Xu, Y., Johnson, T., Zhang, F., Deisseroth, K., and Tracey, W.D. (2007). Nociceptive Neurons Protect Drosophila Larvae from Parasitoid Wasps. Curr. Biol. 17, 2105–2116.
Im, S.H., and Galko, M.J. (2012). Pokes, sunburn, and hot sauce: Drosophila as an emerging model for the biology of nociception. Dev. Dyn. 241, 16–26.
Kavaliers, M. (1988). Evolutionary and comparative aspects of nociception. Brain Res. Bull. 21, 923–931.
Khuong, T.M., Wang, Q.-P., Manion, J., Oyston, L.J., Lau, M.-T., Towler, H., Lin, Y.Q., and Neely, G.G. (2019). Nerve injury drives a heightened state of vigilance and neuropathic sensitization in Drosophila. Sci. Adv. 5, eaaw4099.
Lister, K.C., Bouchard, S.M., Markova, T., Aternali, A., Denecli, P., Pimentel, S.D., Majeed, M., Austin, J.-S., de C Williams, A.C., and Mogil, J.S. (2020). Chronic pain produces hypervigilance to predator odor in mice. Curr. Biol. CB 30, R866–R867.
Nesse, R.M. (2019). The smoke detector principle: Signal detection and optimal defense regulation. Evol. Med. Public Health 2019, 1–1.
Walters, E.T., and Williams, A.C. de C. (2019). Evolution of mechanisms and behaviour important for pain. Philos. Trans. R. Soc. B Biol. Sci. 374, 20190275.
Xu, S.Y., Cang, C.L., Liu, X.F., Peng, Y.Q., Ye, Y.Z., Zhao, Z.Q., and Guo, A.K. (2006). Thermal nociception in adult Drosophila: behavioral characterization and the role of the painless gene. Genes Brain Behav. 5, 602–613.
Journal of Biological Sciences at Rutgers Camden (JBS) is licensed under a Creative Commons Attribution-NonCommercial-ShareAlike 4.0 International License