Cindy Do, Pamela Phan, Kenia Sanchez, and Ozlem Ulutas
Department of Biology, Rutgers University, Camden NJ 08102
Abstract
The adverse health effects of prenatal exposure to tobacco smoke have been studied and acknowledged for many years, representing a major public health concern. The use of nicotine containing products could lead to obstetrical, fetal, and developmental complications, as well as an increased risk of adverse health consequences in adult offspring. A Drosophila melanogaster model demonstrated that when the maternal generation ceases nicotine use prior to pregnancy, the nicotine present in the mother’s system continues to cause anatomical changes within the offspring. The connection between maternal nicotine use and its effect on the offspring’s functional capacity is still a novel research topic. We aimed to explore how maternal nicotine exposure prior to pregnancy influences the first generation’s (F1) functional capacity by studying their respiratory rates. To this end, virgin female D. melanogaster flies were exposed to either distilled water (dH2O), or nicotine vapor once per hour for a total of eight times. Following the last exposure, each of the treatment groups were mated with non-exposed males. The F1 male generation flies were further collected, and their respiratory rates were analyzed. We demonstrated that maternal exposure to nicotine negatively affects the functional capacity of the offspring within the respiratory system as it causes a decrease in their respiratory rates. We anticipate that this research can act as a foundation to help test for additional health effects on the offspring of maternal nicotine use prior to pregnancy.
Introduction
Millions of people worldwide suffer from addictions, preventable diseases, and premature death through the use of tobacco cigarettes (Benowitz, 2010). Cigarette smoking has been proven to be physically addictive due to nicotine, an addictive central nervous system stimulant naturally found in tobacco leaves and defined as a chemical and plant alkaloid. Nicotine and its metabolites are the most specific markers of damage from smoking, which makes it necessary to further study the effects caused by this chemical (Leone et al., 2010). The use of nicotine-based products such is increasing at exponential rates, as these products are heavily advertised as a healthier and safer alternative to tobacco cigarettes, targeting vulnerable populations, such as pregnant women or women who are planning to be pregnant (Association, 2022). Maternal nicotine use leads to an increased risk for poor perinatal outcomes, including low birth weight, congenital abnormalities, and stillbirths (Kurti et al., 2017).
In a model with D. melanogaster, it was demonstrated that maternal nicotine exposure negatively affects the size, weight, and tracheal development of the F1 generation through epigenetic mechanisms (El-Merhie et al., 2021). Scientific researchers have primarily focused on the correlation between maternal use and anatomical changes, rather than functional changes. There is still rather limited knowledge of the functional consequences nicotine may cause within offspring, as the intergenerational effects are only slightly studied. Additionally, recent evidence suggests that the F1 generation would still encounter negative effects, despite the maternal generation ceasing usage prior to pregnancy. Although this is still a novel research topic, it is essential that healthcare providers understand the risks of nicotine use prior to and during pregnancy, as it is relevant to the offspring’s overall health and ability to function. Thus, to focus on one specific functional aspect of the offspring, we strived to study how the respiratory rate will be affected in the F1 generation. By better understanding the effects of maternal nicotine exposure and its role in respiration among the F1 generation, the use of nicotine products may be reduced, and the negative effects of nicotine on future generations of offspring may be prevented.
To study this, we designed an experiment to assess the impact of maternal nicotine exposure on the F1 generation’s respiration rate within D. melanogaster, a common fruit fly that has played a crucial role in research for scientists over the past few years due to their biological makeup. D. melanogaster is a human disease model organism that has much of the same biological makeup as humans, since nearly 60% of the D. melanogaster genome is homologous to that of humans. About 75% of the genes associated with human disease have homologs in flies, thus making them effective model organisms for the study of any human disease mechanisms or health defects (Mirzoyan et al.). Moreover, fruit flies are a common tool in biological research due to their size and rapid reproduction rate. Fruit flies can develop in a short period of time in a laboratory setting. Thus, this allows researchers to carry out experiments and collect data within a relatively short period of time. Additionally, other advantages include its low cost, which makes its level of obtainability higher overall.
We hypothesized that maternal nicotine exposure would lead to a decrease in the respiratory rate of the F1 generation of D. melanogaster. Studying the relationship between nicotine use and respiratory rate develops a path for researchers to establish experiments that can more accurately address the harm of use. Future researchers may be able to develop treatments that specifically target this mechanism, providing additional resources to patients with diseases caused by nicotine.
Materials & Methods
D. melanogaster Strain and Culture
Wild-type Oregon-R D. melanogaster flies were obtained from Dr. Lee’s lab at Rutgers University-Camden. The stock was cultured and maintained at room temperature (25°C) and a humidity of 65-75% at a 12:12 hour light-to-dark cycle. The flies were nourished using a food mixture including purified water, agar, potassium sodium, sucrose, calcium chloride, dextrose, deactivated yeast, and corn. After the Oregon-R adult flies had produced larvae, they were transferred to different vials to separate them from the emerging flies. After eclosion, the period of the emergence of adults from their pupal cases, the new adults within the vials were further divided into two groups: female virgins and male virgins. The virgin flies were collected every four hours and transferred into vials with fresh medium, in which they were isolated in groups by gender. During this isolation period, we were able to monitor the vials for any potential larvae formation. If a vial had contained larvae, this would serve as an indication that the flies in that vial were not virgins, due to inaccurate gender separation, in which we would discard them. This process ensured that the flies collected to be used in our experiment, were in fact virgins.
Nicotine Exposure
The male D. melanogaster from the parental generation were split into two groups: the group exposed to 20 µL of 2.5 mM nicotine, and the group exposed to dH2O (Fig. 1). We were able to transport the flies between their vials containing their food source and the exposure chambers by utilizing a tubular suction device. The groups were placed into separate 250 mL exposure chambers, where the top was sealed with a piece of cheesecloth, along with a layer of parafilm to prevent the potential vapor from escaping (Fig. 2A). To separate the fruit flies from the heated, coiled nichrome wire, a cheesecloth layer was secured near the middle portion of the chamber. This also further prevented the fruit flies from undergoing any form of heat stress. In their respective treatments, either dH2O or nicotine was pipetted onto a coiled nichrome wire connected to a power supply (3.7 V, 1.9 A) which was heated, causing either solution to vaporize. The exposure was repeated once per hour for 2 minutes, for a total of eight times. Following the exposures, their respiratory rates were further analyzed and measured with respirometers and carbon dioxide (CO2) measurement chambers (Fig. 2).
Meanwhile, the female virgin D. melanogaster from the parental generation were split into two groups: the group exposed to 20 µL of 2.5 mM nicotine, and the group exposed to 20 µL of dH2O (Fig. 1). We followed the same procedure for the exposures within the female virgins, as conducted with the male D. melanogaster. Following the last exposures with the female virgin fruit flies, they were transferred to individual vials to be mated with non-exposed male fruit flies, depending on the treatment received. The F1 generation from maternal nicotine exposure is the experimental group, while the F1 generation from maternal dH2O exposure is the control group. After the female flies have laid eggs of the F1 generation, the parental generation flies were relocated to a new vial to isolate the F1 generation. After the F1 generation had transformed into adults, their respiratory rates were further analyzed and measured with respirometers and CO2 measurement chambers (Fig. 2).
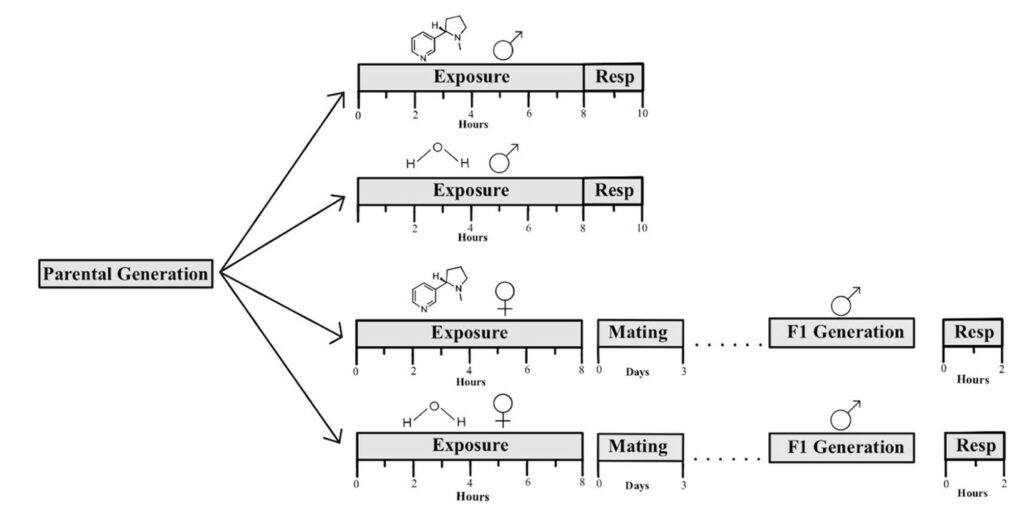
Figure 1. Experimental timeline schematic for animal experiments. The top two timelines depict the 8-hour treatments within the male parental generation, followed by the 2-hour measurement of CO2 production through the use of respirometers. The bottom two timelines depict the 8-hour treatments within the female parental generation, followed by mating for 3 days. Once the males of the F1 generation transformed into adults, a 2-hour measurement of CO2 production was conducted.
Respirometer
The respirometers were built with 1,000 μL pipette tips and inserted into 50 μL capillary tubes. Then, they were further stabilized with glue, which took approximately 24 hours to dry. Two small pieces of cotton were placed inside the 1,000 μL pipette tips, separated by a small quantity of soda lime (the CO2 absorbent). To transport the fruit flies into the respirometers, they were anesthetized on a cold surface. The F1 generation of fruit flies was sealed in the pipette of the respirometers using parafilm as a barrier between the flies and the plasticine clay on top. The fruit flies were given a total of 5 minutes to awaken and equilibrate in the respirometers. Following this period, the respirometers were placed into a CO2 measurement chamber with their tips submerged in a 900 mL solution of water and food coloring. We allowed the systems to equilibrate for 15 minutes, during which we photographed the chamber. The chamber was also photographed after a total of 2 hours following the equilibration. Since these respirometers serve as a sealed environment, oxygen cannot enter or leave. Over the course of two hours, as the animals were respiring or breathing, they were expelling CO2, which was absorbed by the soda lime, causing a vacuum mechanism to be created, sucking up water to a certain distance on the respirometer, shown within the capillary tubes (Fig. 2C). ∆d indicates the distance the liquid has traveled in the capillary tube, otherwise known as the level of CO2 production (Fig. 2C). To further explain, during cellular respiration, ingested nutrients in the form of glucose and oxygen (O2) are converted to energy in the form of adenosine triphosphate. During this process, CO2 is produced as a byproduct of the reaction (Patel et al., 2022). O2 is obtained through inhalation, and the CO2 generated is then removed from the body through exhalation. Thus, CO2 production levels may serve as a direct indication of respiratory rate.
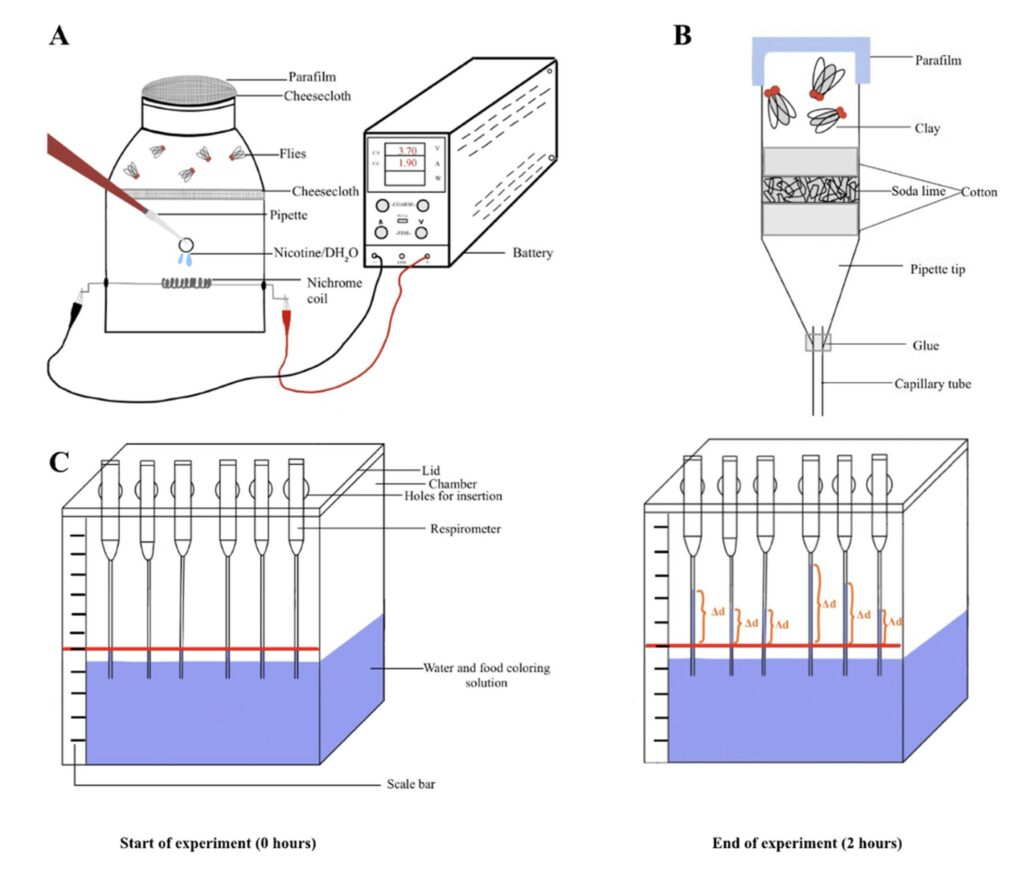
Figure 2. Setup for nicotine exposure and CO2 measurement. (A). Schematic of the nicotine exposure chamber. (B). Schematic showing the construction of the individual respirometer (C). Schematic showing the changes during the experiment. The red lines indicate the reference point (d1), and the orange marks on the right indicate the final position of the solution after 2 hours (d2). Thus, ∆d indicates the distance the liquid has traveled in the capillary tube.
Respirometer Assay
To access and confirm the reliability of the respirometers, a control test is run using wild-type Oregon-R D. melanogaster. This assay measured solution displacement, which is a direct indication of CO2 production. Hypothetically, the total volume of CO2 should increase as the number of fruit flies increases, which is a direct indication of a functioning respirometer (Fig. 2). To accomplish this, three respirometers are tested with increasing numbers of D. melanogaster flies. In order, the respirometers contained zero, three, and five flies. After anesthetizing the flies with an ice pack, they were placed in their respective respirometers at the top of the pipette. The first respirometer served as the atmospheric control or negative control. In this respirometer, we had no D. melanogaster flies present. The respirometers are inserted with the tip of the capillary tube submerged straight down into the solution. Temperature and pressure fluctuations are further eliminated by adding tape to seal the lid and chamber. Following the insertion of the respirometers, the system had equilibrated for a total of 15 minutes. Following this period, photographs were taken to record the data. One image is taken at the start of the experiment, with the second image taken at the end of the experiment after 2 hours. Image J was used to analyze the displacement of the solution among the respirometers using the pixel scaling feature with the assistance of the scale bar along the side of the chamber. The values were then averaged, showing the ± standard error. The data from this assay allowed for the examination of statistically different results for the CO2 production levels within the respective amounts of fruit flies and confirmed the efficacy of the respirometers and chamber.
Analysis of Results
Image J was used to analyze the displacement of the solution among the respirometers using the pixel scaling feature. The change in distance (Δd) between the liquids from their initial levels to the end of the experiment was recorded. Within the respirometer assay, we calculated the amount of CO2 produced (μl/hr*fly or μl/hr*mg ) using the formula:
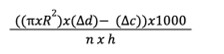
where R represents the radius of the capillary tube in centimeters and Δd represents the distance that the liquid has traveled up the capillary tube of each sample measured in centimeters. Δc represents the distance the liquid has traveled up the capillary tube of the negative control sample (in the absence of flies). n represents the number of flies used, while h represents hours (Yatsenko et al., 2014).
Moreover, the data can also be presented as (μl/hr*mg) by measuring the mass of the flies after performing the assay if there are significant differences in the size of the flies. In our experiment, one D. melanogaster fly weighed 0.65 ± 0.5 mg (n = 16). Following the treatments, we calculated the amount of CO2 produced (μl/hr/mg) within the various groups to normalize the data due to any size differences.
Results
A respirometer assay was conducted to test the viability of the respirometers and measurement chamber. The assay was replicated four times to calculate average values of CO2 production for the flies. When comparing the overall displacement for each of the respirometers, it was found that the respirometers containing three flies had a higher displacement value than the control. However, as anticipated, the respirometers containing five flies produced a significantly higher level of CO2 compared to the respirometers containing only no fruit flies and three fruit flies (Fig. 3A). Additionally, if two or fewer flies were placed in one respirometer, the data was unreliable. Therefore, 3–5 D. melanogaster is the ideal amount for conducting these experiments. The findings of this assay revealed a statistically significant difference in average CO2 levels, thus confirming that the respirometers are functional (Mann-Whitney test; p < 0.05). The respirometers were an effective method of measuring the CO2 production levels within D. melanogaster flies since they showcased a positive correlation between the number of fruit flies and CO2 levels (Fig. 3A).
To analyze whether nicotine exposure leads to any physiological impacts on the respiratory system and flies within the parental generation, we assessed the respiratory rate by analyzing the CO2 production levels. The parental male flies were exposed to 20 µL of 2.5 mM nicotine or dH2O, and their respiratory rates were measured directly after the exposures (Fig. 3B). The data demonstrated a significant difference between the dH2O flies and the nicotine flies. However, the results demonstrate a significant decrease in CO2 production due to the dH2O and not the nicotine (t-test ; p < 0.05).Although the parental generation demonstrated an increase in respiration following nicotine exposure, the F1 generation demonstrated a significant decrease in respiration, as hypothesized (Fig. 3C). To examine a notable difference among the F1 generation’s treatments, an unpaired t-test was performed, which demonstrated that nicotine did in fact lead to a decline in CO2 production in the F1 generation. Our results demonstrate that the transgenerational nicotine effects were more detrimental to the F1 generation than the direct exposure in the parental generation (Fig. 3).
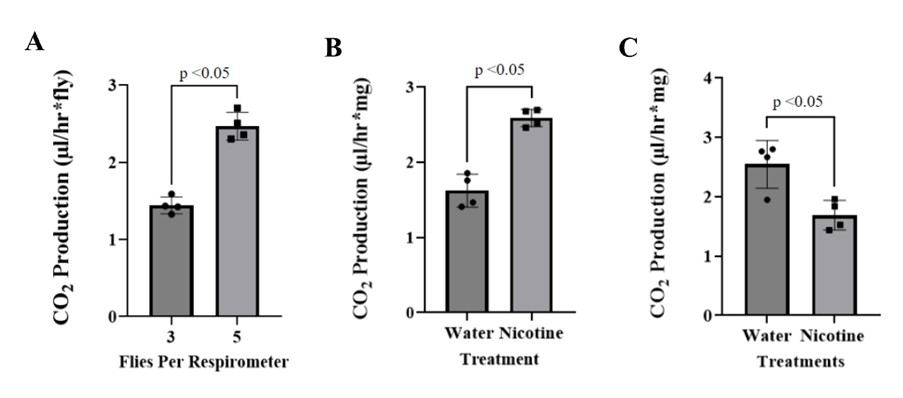
Figure 3. CO2 production by flies. (A). Average volume of CO2 production from the respirometer assay. The standard error bars represent the ± SEM values. The average level of CO2 production within the respirometers of three fruit flies was significantly less than the level of CO2 production within the respirometers of five fruit flies (t-test; p < 0.05, n = 4). (B). Averages of the volume of CO2 production levels of the paternal generation of D. melanogaster flies following respective exposures. (C). Showcases the CO2 production levels of the F1 generation, following their respective maternal treatments with water or nicotine. The experimental data consisted of mean values ± SEM, t-test, p < 0.05, n = 4.
Discussion
Our study investigated the impact of maternal nicotine exposure before mating on the respiratory rate of the F1 generation in D. melanogaster. CO2 production levels in measurement chambers were recorded to assess the influence of nicotine on respiratory rate. Our results indicated that maternal nicotine exposure leads to reduced respiratory rates in adult males of the F1 generation in D. melanogaster (Fig. 3C). Our findings suggest that maternal nicotine use can produce enduring health consequences for offspring, aligning with previous research studies and demonstrating long-lasting biological effects (Hawkey et al., 2019).
The parental generation initially served as a control for our F1 generation observations. However, our data revealed a significant increase in respiratory rate when the parental generation was directly exposed to 2.5 mM nicotine compared to dH2O (Fig. 3B). This finding confirms that firsthand nicotine exposure induces physiological effects on respiration in flies. Firsthand nicotine exposure is known to elevate blood pressure, heart rate, and myocardial contractility by stimulating sympathetic neurotransmission through nicotinic acetylcholine receptors (Haass and Kübler, 1997). Nicotine activates the release of norepinephrine, leading to increased heart rate and contractility (Haass and Kübler, 1997). A rat model demonstrated that microinjections of nicotine into preBötC, a group of excitatory inspiratory neurons, increased respiratory frequency and reduced inspiratory bursts (Shao and Feldman, 2001). These findings collectively indicate that firsthand nicotine exposure influences excitatory neurotransmission, impacts inspiratory neurons, and heightens respiratory-related motor activity (Shao and Feldman, 2001).
While the parental generation demonstrated increased respiration, the F1 generation exhibited decreased respiration, which cannot be attributed to differences in developmental size as we normalized the data (Fig. 3). This decline in respiration suggests an epigenetic mechanism involving gene regulation. Epigenetics refers to modifications in gene expression rather than changes in the genetic code itself (Howie et al., 2019).HDAC2, an enzyme involved in histone acetylation patterns, is inhibited by frequent nicotine exposure, leading to epigenetic changes that reduce gene expression (Volkow, 2011). Altered histone acetylation patterns may result in epigenetic modifications (Géranton and Tochiki, 2015). Histone acetylation levels tend to rise when HDAC2 is broken down, or its activity is decreased, altering gene expression. Studies have linked lower HDAC2 activity to changes in gene expression association with various illnesses (Chen et al., 2015). The epigenetic changes can be transgenerational through the germline (Bohacek and Mansuy, 2013). In a mice model, maternal electronic cigarette exposure led to DNA methylation changes and altered lung cytokine expression in offspring. Offspring exposed to electronic cigarette liquid containing 18 mg/ml nicotine exhibited doubled global DNA methylation levels in their developing lungs, when compared to the control offspring exposed to room air (Nguyen et al., 2018). In a D. melanogaster model, maternal nicotine exposure has been shown to produce detrimental effects on fertility and various characteristics of the F1 generation, including size, weight, and tracheal development, through epigenetic mechanisms (El-Merhie et al., 2021). Since fruit flies carry eggs rather than babies, the nicotine exposure conducted in the experiment is not implemented within an in-utero exposure system. This further solidifies the idea that the observed effects are attributed to epigenetic changes.
Our study had certain limitations in terms of time and resources. It would have been more feasible to obtain more conclusive results if the F1 offspring descended from the same parental father. Each F1 offspring descended from a different lineage, making it difficult to compare the results conclusively. Parental likeness would fortify the conclusion that the decrease in respiratory rate within the F1 generation resulted from maternal nicotine exposure rather than differing genes or external influences on each individual offspring. Additionally, variability in the construction of the individually made respirometers may have contributed to errors. Certain exposures resulted in outlier values, indicating non-functioning respirometers, potentially due to inadequate glue or clay for airtightness, or insufficient space between the cotton and the plug for the D. melanogaster flies. In future studies, these variations may be limited with improved equipment. Furthermore, conducting experiments on a larger number of flies over an extended period would provide more data from a greater number of trials, thereby strengthening the efficacy of the conclusions.
Referring to evidence in the literature, past studies have indicated that the concentrations of nicotine used are subthreshold concentrations for mortality with significant psychological and functional effects in D. melanogaster (El-Merhie et al., 2021). The D. melanogaster flies have homologs of the human cytochrome P450 CYP1A1, which is necessary for xenobiotic metabolism (El-Merhie et al., 2021). However, a limitation of our study is the difficulty in accurately predicting how the dose of nicotine administered to the D. melanogaster flies correlates with human exposure. Nonetheless, D. melanogaster flies were used in our study to pose as models of potential health defects in human offspring. Further studies within other model organisms can further solidify the nicotine concentration to body ratio, which can be applied to human health. This study was valuable for further studying the impact of maternal nicotine exposure on the respiratory rate of the F1 generation. Specifically, it focused on nicotine and its approach to decreasing respiratory levels in offspring. The outcome of this current study revealed that maternal nicotine exposures administered prior to mating produce a decrease in the functional capacity of the offspring. Although the null hypothesis was rejected, future long-term research could further study different functional aspects of the offspring that may be affected to try and develop an understanding of the mechanistic approach behind this epigenetic effect.
To further explore to what extent maternal nicotine exposures can produce a decrease in respiratory levels within the offspring, a potential experimental design for future studies could involve exposing virgin female flies to nicotine once per hour for a total of eight times. Following the exposures, the virgin female flies are isolated for increasing periods of time. In order, the flies may be isolated for zero, one, two, three, four, and five days. Following the isolation period, the females will mate and produce offspring, enabling the study of differences in respiratory rate, with the potential for females to abstain from smoking and prevent health defects in their offspring. Furthermore, a survival assay of the F1 flies could be conducted, recording the number of deceased flies until no survivors remain to observe their survival rates, which may be influenced by the duration of maternal isolation before pregnancy. The results of this proposed experiment would contribute to our understanding of how maternal nicotine exposure impacts offspring functional capacity and survival.
Our results further demonstrate that maternal exposure to nicotine is associated with fetal abnormalities in flies. Currently, there is still rather limited knowledge on the long-term functional consequences nicotine may cause within human offspring due to maternal use, as the intergenerational effects are only slightly studied. We hope our findings highlight the importance of human studies investigating the effects of maternal nicotine use on the respiratory health of offspring and their overall functional capacity. Further, the prominence of such substance addictions within our society reiterates the importance of delving into the components of this research and its potential influence on patients and their offspring in the future.
Acknowledgments
We would like to express our gratitude to Dr. Kwangwon Lee and Dr. Nathan Fried for the endless support and guidance that they provided throughout the duration of the project. We would also like to thank Rutgers University – Biology Department for providing us with resources and funding.
References
Association, A.L. Tobacco Use among Children and Teens.
Benowitz, N.L. (2010). Nicotine Addiction. N. Engl. J. Med. 362, 2295–2303. https://doi.org/10.1056/nejmra0809890.
Bohacek, J., and Mansuy, I.M. (2013). Epigenetic Inheritance of Disease and Disease Risk. Neuropsychopharmacology 38, 220–236. https://doi.org/10.1038/npp.2012.110.
CDCTobaccoFree (2022). Health Effects of Cigarette Smoking.
Chen, G., Guan, F., Lin, H., Li, L., and Fu, D. (2015). Genetic analysis of common variants in the HDAC2 gene with schizophrenia susceptibility in Han Chinese. J. Hum. Genet. 60, 479–484. https://doi.org/10.1038/jhg.2015.66.
El-Merhie, N., Krüger, A., Uliczka, K., Papenmeier, S., Roeder, T., Rabe, K., Wagner, C., Angstmann, H., and Krauss-Etschmann, S. (2021). Sex dependent effect of maternal e-nicotine on F1 Drosophila development and airways.
Géranton, S.M., and Tochiki, K.K. (2015). Could targeting epigenetic processes relieve chronic pain states? Curr. Opin. Support. Palliat. Care 9, 138–146. https://doi.org/10.1097/SPC.0000000000000127.
Haass, M., and Kübler, W. (1997). Nicotine and sympathetic neurotransmission. Cardiovasc. Drugs Ther. 10, 657–665. https://doi.org/10.1007/BF00053022.
Hawkey, A.B., White, H., Pippen, E., Greengrove, E., Rezvani, A.H., Murphy, S.K., and Levin, E.D. (2019). Paternal Nicotine Exposure in Rats Produces Long-lasting Neurobehavioral Effects in the Offspring. Neurotoxicol. Teratol. 74, 106808. https://doi.org/10.1016/j.ntt.2019.05.001.
Howie, H., Rijal, C.M., and Ressler, K.J. (2019). A review of epigenetic contributions to post-traumatic stress disorder. Dialogues Clin. Neurosci. 21, 417–428. https://doi.org/10.31887/DCNS.2019.21.4/kressler.
Kurti, A.N., Redner, R., Lopez, A.A., Keith, D.R., Villanti, A.C., Stanton, C.A., Gaalema, D.E., Bunn, J.Y., Doogan, N.J., Cepeda-Benito, A., et al. (2017). Tobacco and nicotine delivery product use in a national sample of pregnant women. Prev. Med. 104, 50–56. https://doi.org/10.1016/j.ypmed.2017.07.030.
Leone, A., Landini, L., and Leone, A. (2010). What is tobacco smoke? Sociocultural dimensions of the association with cardiovascular risk. Curr. Pharm. Des. 16, 2510–2517. https://doi.org/10.2174/138161210792062948.
Mai, Z., Lei, M., Yu, B., Du, H., and Liu, J. (2014). The Effects of Cigarette Smoke Extract on Ovulation, Oocyte Morphology and Ovarian Gene Expression in Mice. PLoS ONE 9, e95945. https://doi.org/10.1371/journal.pone.0095945.
Mirzoyan, Z., Sollazzo, M., Allocca, M., Valenza, A., Grifonia, D., and Bellosta, P. Drosophila melanogaster: A Model Organism to Study Cancer. 2019 https://doi.org/10.3389/fgene.2019.00051.
Nguyen, T., Li, G.E., Chen, H., Cranfield, C.G., McGrath, K.C., and Gorrie, C.A. (2018). Maternal E-Cigarette Exposure Results in Cognitive and Epigenetic Alterations in Offspring in a Mouse Model. Chem. Res. Toxicol. 31, 601–611. https://doi.org/10.1021/acs.chemrestox.8b00084.
Patel, S., Miao, J.H., Yetiskul, E., Anokhin, A., and Majmundar, S.H. (2022). Physiology, Carbon Dioxide Retention. In StatPearls, (Treasure Island (FL): StatPearls Publishing), p.
Products, C. for T. (2020). Harmful and Potentially Harmful Constituents in Tobacco Products and Tobacco Smoke: Established List. FDA.
Products, C. for T. (2022). Nicotine Is Why Tobacco Products Are Addictive. FDA.
Services, D. of H.& H. Smoking – effects on your body (Department of Health & Human Services).
Shao, X.M., and Feldman, J.L. (2001). Mechanisms underlying regulation of respiratory pattern by nicotine in preBötzinger complex. J. Neurophysiol. 85, 2461–2467. https://doi.org/10.1152/jn.2001.85.6.2461.
Spindel, E.R., and McEvoy, C.T. (2016). The Role of Nicotine in the Effects of Maternal Smoking during Pregnancy on Lung Development and Childhood Respiratory Disease. Implications for Dangers of E-Cigarettes. Am. J. Respir. Crit. Care Med. 193, 486–494. https://doi.org/10.1164/rccm.201510-2013PP.
Volkow, N.D. (2011). Epigenetics of Nicotine: Another Nail in the Coughing. Sci. Transl. Med. 3, 107ps43. https://doi.org/10.1126/scitranslmed.3003278.
Yatsenko, A.S., Marrone, A.K., Kucherenko, M.M., and Shcherbata, H.R. (2014). Measurement of metabolic rate in Drosophila using respirometry. J. Vis. Exp. JoVE e51681. https://doi.org/10.3791/51681.
Journal of Biological Sciences at Rutgers Camden (JBS) is licensed under a Creative Commons Attribution-NonCommercial-ShareAlike 4.0 International License